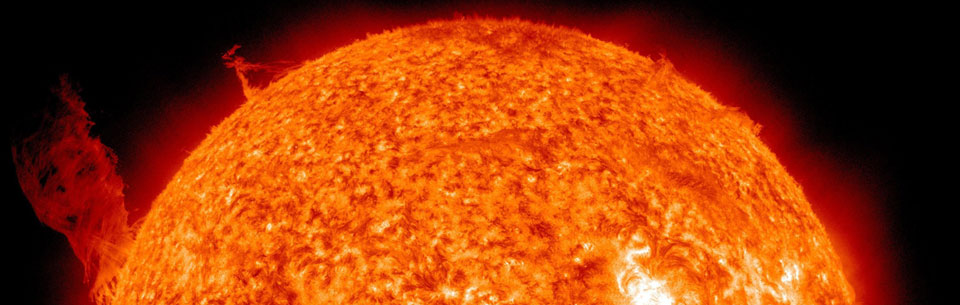
Inertial Confinement Fusion
Magnetized Liner Inertial Fusion (MagLIF)
Centered on magnetically driven implosions
Fusion: The ultimate energy source
Einstein’s famous equation, E = mc2, tells us that a small amount of mass can be converted into a large amount of energy. This powerful equation is at the center of fusion energy – the idea that light nuclei, e.g. deuterium and tritium (isotopes of hydrogen) can be smashed together to form particles, e.g. a neutron and a helium nuclei, of even smaller combined mass. The mass that is lost is converted to kinetic or “motion” energy of the new particles according to Einstein’s equation, which can then be used for producing electricity.
Of course, there is a major challenge in making fusion work. Significant amounts of fusion reactions can only occur when the hydrogen nuclei are very close together, around 1/10 of a trillionth of a meter (10-13 m) apart. But since the deuterium and tritium nuclei are both positively charged, the nuclei try to “push” each other away due to electrical repulsion. In order to get the nuclei close enough to each other and make fusion a reality, we need to do two things. First, we need to get enough hydrogen nuclei close together by compressing the hydrogen fuel. By increasing the density of the hydrogen fuel through compression, we can increase the likelihood of fusion reactions to occur. And secondly, we need to heat the fuel to hot enough temperatures, >10 million degrees Celsius, so that nuclei can overcome the electrical repulsion. This illustration shows hydrogen fuel in fusion relevant conditions.
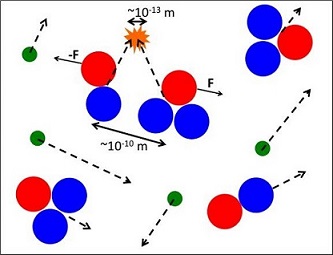
A picture of a hydrogen fuel with deuterium and tritium in fusion relevant conditions. Protons are shown in red, neutrons are in blue, and electrons are in green. The fuel has been compressed sufficiently dense so that the average distance between nuclei is ~ 10-10 m. In a high temperature fuel undergoing fusion reactions, the velocities (shown as dashed arrows) of the nuclei are sufficiently large in magnitude to overcome the electric repulsion forces, F, between protons in the nuclei. When deuterium and tritium nuclei get to within ~10-13 m of each other, a fusion reaction (shown in orange) can occur.
Inertial Confinement Fusion
Inertial Confinement Fusion (ICF) achieves fusion conditions by rapidly compressing and heating a small quantity of fusion fuel. This results in a very high fuel pressure, which inevitably causes the fuel to disassemble. In ICF, the desired fusion burn must be completed before this fast disassembly occurs.
Scientists at Sandia have now demonstrated experimentally that an ICF concept called Magnetized Liner Inertial Fusion (MagLIF) is capable of achieving thermonuclear fusion conditions on the Z machine (see sidebar). Additionally, computer simulations indicate that MagLIF could eventually achieve scientific breakeven on the Z machine. Researchers study these fusing plasmas for various stockpile stewardship and fusion energy applications.
Three Stages of MagLIF
(Left) A centimeter-tall cylindrical metal shell (the liner) is filled with deuterium gas (the fusion fuel). A large electrical current running from top to bottom along the shell’s outer surface produces a magnetic field that wraps around the outside of the shell (circular grey lines). The resulting powerful electromagnetic force causes the shell to implode and compress the deuterium fuel contained within the shell.
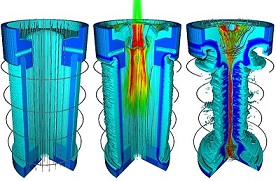
An axial magnetic field (vertical grey lines) helps keep the fuel hot during compression. (Middle) A laser quickly heats and ionizes the fuel into a plasma state just as the implosion begins. (Right) The liner then implodes, which compresses and further heats the fuel to fusion relevant temperatures (above 10 million degrees) and densities (above 1 gram per cubic centimeter, which is the density of water at room temperature). (M. R. Gomez et al., Phys. Rev. Lett. (2014))
Long-term vision
The long-term goal of the pulsed-power based, magnetically driven target approach is to achieve high single-shot yields (0.5-1 GJ per shot). This capability would support key objectives for the Stockpile Stewardship Program run by the National Nuclear Security Administration. If this approach is successful, it may be possible to achieve these yields from targets absorbing up to 10 MJ in a laboratory pulsed power facility with a stored energy of roughly 130 MJ. Such a facility would be substantially cheaper, and not as complex, than the corresponding pulsed power facility required for producing comparable yields from x-ray driven capsule targets.
We are currently developing a credible case for a next-step facility that might be capable of ignition. To clarify what we mean by ignition, here we define it as the point where the thermonuclear yield equals the energy absorbed by the target. Our target scaling designs suggest that this is approximately 3-5 MJ for MagLIF targets. The 24 MJ, 80-TW Z facility today couples about 0.5 MJ to targets.
Our present estimates suggest that it would take a 50 MJ, 300-TW facility to reach ignition. In addition to studying the physics and scaling of MagLIF targets, we are attempting to develop next generation pulsed power technology based on the Linear Transformer Driver (LTD). This architecture is fundamentally different than the traditional “Marx-based” architecture used for the Z facility and offers several advantages including the fact that it is twice as energy efficient (explaining why the next-step facility needs to be 50 MJ instead of 100 MJ).
Our predictions for the facility size needed to reach ignition are based on the best currently available models for the coupling between that driver and the target, the coupling of high-energy lasers to the target, the initiation and evolution of implosion instabilities during the acceleration stage, and the evolution of instabilities and mix during the deceleration stage. All of these effects occur in the presence of strong azimuthal and axial magnetic fields that alter the implosions and particle transport. In short, a lot of science must be done to understand how good these models really are. Ultimately, it is likely that the best demonstration of our understanding of these models will be showing that we can obtain stagnation plasma conditions consistent with our predictions at different scales.
First Trace Tritium Experiment Conducted on Z Machine
Sandia’s researchers have opened a new chapter in their 20-year journey by introducing tritium to their targets’ fuel.
Read the fascinating article, Tritium Introduced in Fusion Experiments at Sandia from Lab News – Oct 28, 2016.