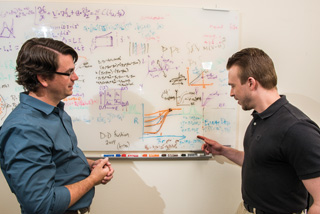
Sandia researchers have produced a significant output of fusion neutrons, using a method fully functioning for only little more than a year.
The experimental work is described in a paper to be published in the Sept. 24 Physical Review Letters (PRL) online. A theoretical PRL paper to be published on the same date helps explain why the experimental method worked. The combined work demonstrates the viability of the novel approach.
Says senior manager Dan Sinars (1680), “We are committed to shaking this [fusion] tree until either we get some good apples or a branch falls down and hits us on the head.” He expects the project, dubbed MagLIF, for magnetized liner inertial fusion, will be “a key piece of Sandia’s submission for a July 2015 NNSA review of the national Inertial Confinement Fusion Program.”
MagLIF uses a laser to preheat hydrogen fuel, a large magnetic field to squeeze the fuel, and another separate magnetic field to keep charged atomic particles from leaving the scene.
Inertial confinement fusion creates nanosecond bursts of neutrons, ideal for creating data to plug into supercomputer codes that test the safety, security, and effectiveness of the US nuclear stockpile. Down the road, if the individual fusion pulses can be sequenced like an automobile’s cylinders firing, the method could be useful as an energy source.
It only took two magnetic fields and a laser, focused on a small amount of fusible material called deuterium (hydrogen with a neutron added to its nucleus), to produce a trillion fusion neutrons (neutrons created by the fusing of atomic nuclei). Had tritium (which carries two neutrons) been included in the fuel, scientific rule-of-thumb says that 100 times more fusion neutrons would have been released. (That is, the actual release of 10 to the 12th neutrons would be upgraded, by the more reactive nature of the fuel, to 10 to the 14th neutrons.)
Technique is still a toddler
Even with this larger output, to achieve break-even fusion — as much power out of the fuel as placed into it — 100 times more neutrons (10 to the 16th) still would have to be produced. The gap is sizable, but the technique is a toddler, with researchers still involved in figuring out the simplest measures: how thick or thin key structural elements of the design should be, and the relation between the three key aspects of the approach — the two magnetic fields and the laser.
The first Sandia paper, “Experimental Demonstration of Fusion-Relevant Conditions in Magnetized Liner Inertial Fusion,” [MagLIF] by lead authors Matt Gomez (1683), Steve Slutz, and Adam Sefkow (both 1684), describes a fusion experiment remarkably simple to visualize. The deuterium target atoms are placed in a long thin tube called a liner. A magnetic field from two pancake-shaped Helmholtz coils above and below the liner creates an electromagnetic curtain that prevents charged particles, both electrons and ions, from escaping. The extraordinarily powerful magnetic field of Sandia’s Z machine then crushes the liner like an athlete crushing a soda can, forcefully shoving atoms in the container into more direct contact. As the liner begins to be crushed, a laser beam preheats these deuterium atoms, infusing them with energy to increase the chance of them fusing at the end of the implosion. (A nuclear reaction occurs when an atom’s core is combined with that of another atom, releasing large amounts of energy from a small amount of source material. That outcome is important in stockpile stewardship and, eventually, in civilian energy production.) Trapped energized particles including fusion-produced alpha particles (two neutrons, two protons) also help maintain the temperature of the reaction at a high level.
“On a future facility, trapped alpha particles would further self-heat the plasma and increase the fusion rate, a process required for break-even fusion or better,” says Adam.
The actual MagLIF procedure follows this order: The Helmholtz coils are turned on for a few thousandths of a second. Within that relatively large amount of time, a 19-megaAmpere electrical pulse from Z, with its attendant huge magnetic field, fires for about 100 nanoseconds (less than a millionth of a second), with a power curve that rises to a peak and then falls in intensity. Just after the 50-nanosecond mark, near the current pulse’s peak intensity, the laser, called Z-Beamlet, fires for several nanoseconds, warming the fuel.
Slower pace = More fusible reactions
According to the paper’s authors, the unusual arrangement of using magnetic forces both to collapse the tube and simultaneously insulate the fuel, keeping it hot, means that researchers could slow down the process of creating fusion neutrons. What had been a precipitous process using X-rays or lasers to collapse a small unmagnetized sphere at enormous velocities of 300 kilometers per second, can happen at about one-quarter speed at a much more “modest” 70 km/sec. (Modest only comparatively; the speed is about six times greater than that needed to put a satellite in orbit.) The slower pace allows more time for fusible reactions to take place. The more benign implosion also means fewer unwanted materials from the collapsing liner mix into the fusion fuel, which would cool it and prevent fusion from occurring. By analogy, a child walking slowly in the ocean’s shallows stirs less mud than a vigorously running child.
Commenting on these phenomena, Sandia senior scientist Mike Campbell (1200) says, “This experiment showed that fusion will still occur if a plasma is heated by slow, rather than rapid, compression. With rapid compression, if you mix materials emitted from the tube’s restraining walls into the fuel, the fusion process won’t work; also, increased acceleration increases the growth of instabilities. A thicker can [tube] is less likely to be destroyed when contracted, which would dump unwanted material into the deuterium mix, and you also reduce instabilities, so you win twice.”
Says Matt more technically, “We demonstrated that the requirements for inertial confinement fusion can be dramatically reduced using insulating magnetic fields and laser pre-heating of the fuel. This allows us to substantially reduce the required implosion velocity of the target, which allows us to use targets that are more robust to instabilities. The magnetic field also reduces the fuel density requirement by several orders of magnitude.”
Besides the primary deuterium fusion neutron yields, the team’s measurements also found a smaller secondary deuterium-tritium neutron signal that was about a hundred-fold larger than what would have been expected without magnetization, providing a smoking gun for the existence of extreme magnetic fields.
The question had remained whether it was indeed the secondary magnetic field that caused the 100-fold increase in this additional neutron pulse, or some other,
still unknown cause. Fortunately, the pulse has a distinct nuclear signature arising from the interaction of tritium nuclei as they slow down and react with the primary deuterium fuel. This interaction, carrying with it a fingerprint of the influence of the magnetic field on the fusion process, was detected by the sensors of Sandia researchers.
A path to ‘high gain’ fusion conditions
That is the subject of the theoretical paper “Understanding fuel magnetization and mix using secondary nuclear reactions in magneto-inertial fusion.” Using simulations, Paul Schmit (1684), Patrick Knapp (1688), et al confirmed the existence and effect of extreme magnetic fields via simulations. Their calculations showed that the tritium nuclei would be encouraged by these magnetic fields to move along tight helical paths. This confinement increased the probability of subsequently fusing with the main deuterium fuel.
Says Paul, “This dramatically increases the probability of fusion. That it happened validates a critical component of the MagLIF concept as a viable pathway forward for fusion. Our work has helped show that MagLIF experiments are already beginning to explore conditions that will be essential to achieving high yield and/or ignition in the future.”
The foundation of Sandia’s MagLIF work is based on work led by Steve.
In a 2010 Physics of Plasmas article, Steve showed that a tube enclosing preheated deuterium and tritium, crushed by the large magnetic fields of the 25-million-ampere Z machine and a secondary magnetic field, would yield slightly more energy than is inserted into it.
A later simulation, published January 2012 in Physical Review Letters by Steve and Roger Vesey (1684), showed that a more powerful accelerator generating 60 million amperes or more could reach “high-gain” fusion conditions, where the fusion energy released greatly exceeds (by more than 1,000 times) the energy supplied to the fuel.
A paper led by Adam et al, published July 24 in Physics of Plasmas, further explicated and designed the experiments based on predictions made in Steve’s earlier paper.
But, says Mike Campbell, “There is still a long way to go.”